Introduction
Plasmonics is like a superpower for light! Imagine tiny particles on a surface that can especially dance with light. These particles, called surface plasmons, are found at the meeting point of metals and other materials.
What makes them fascinating is that they allow us to control and use light in ways we couldn’t before. It’s like having a secret language with light, and scientists are using this to create super-accurate sensors and tiny devices. So, plasmonics is all about playing with light on a really, really small scale. It’s like having a magical tool to make cool gadgets that can do amazing things!
Define Plasmonics
Plasmonics is a branch of physics and nanotechnology that focuses on the study and manipulation of surface plasmons, which are collective oscillations of free electrons at the interface between a conductor (typically a metal) and a dielectric material. These surface plasmons can be harnessed and controlled to manipulate light at the nanoscale. Plasmonics involves the interaction between electromagnetic field and these surface plasmons, leading to various optical phenomena and applications. This field is particularly important in developing advanced technologies such as sensors, imaging devices, and other nanophotonic devices due to its ability to confine and enhance electromagnetic fields on a scale much smaller than the wavelength of light.
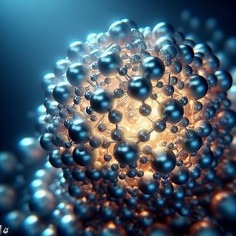
Basics of Plasmonics
A. Characteristics
Plasmons are like energetic ripples or waves created by the synchronized movement of free electrons on the surface of a metal or a conductive material. They are a unique way that electrons collectively interact with light at a tiny scale, typically at the nanometer level.
Characteristics:
1. Collective Oscillation: Plasmons involve the synchronized, cooperative movement of many electrons together, creating a collective behavior.
2. Nanoscale Interaction: These electron dances happen at an incredibly small scale, often at the nanometer level, allowing us to manipulate light in ways not possible with larger materials.
3. Enhanced Fields: Plasmons create intense electric fields near the surface, which can boost the interaction between light and matter, leading to enhanced sensitivity in various applications.
4. Sensitive to Wavelengths: The behavior of plasmons is strongly influenced by the wavelength of the incoming light. This sensitivity makes them valuable for applications like sensing and detecting.
5. Localized and Propagating Plasmons: Plasmons can be localized to a specific region (staying close to where they originated) or propagated across the material, depending on the structure and conditions.
B. Types of Plasmonics
1. Surface Plasmon Resonance (SPR):
– Interaction between light and electron waves at the surface of a metal.
– Used in sensors to detect changes in the refractive index of nearby substances.
2. Localized Surface Plasmons (LSP):
– Concentrated oscillations of electrons on small metallic nanoparticles.
– Produce strong, localized electromagnetic fields, useful in sensing and imaging.
3. Propagating Surface Plasmons (PSP):
– Electromagnetic waves traveling along the interface of a metal and dielectric.
– Applied in technologies like waveguides for guiding and manipulating light.
4. Dark Plasmons:
– Excitations with weak interaction with light, often challenging to detect.
5. Hot Electrons:
– High-energy electrons generated by plasmonic interactions.
– Utilized in photodetectors and solar cells for efficient energy conversion.
6. Gap Plasmons:
– Plasmonic modes arising in the narrow gaps between metallic nanostructures.
– Employed in enhancing light-matter interactions for various applications.
Understanding these types of plasmons allows researchers to tailor their properties for specific applications in areas like sensing, imaging, and energy conversion.
Surface Plasmon Resonance (SPR)
Surface Plasmon Resonance (SPR) is a fascinating phenomenon occurring at the interface of a metal and a dielectric material, often utilized in sensing applications. Here’s a breakdown.
1. Location of Action:
– Takes place at the surface of a thin metal film, commonly gold or silver.
2. Interaction with Light:
– When light hits this metal-dielectric interface at a specific angle, it excites surface plasmons.
3. Resonance Condition:
– SPR occurs under specific conditions where the energy from incident light matches the energy required to excite the surface plasmons.
4. Refractive Index Sensing:
– The resonance angle is highly sensitive to changes in the refractive index of the material near the metal surface.
5. Sensing Applications:
– Utilized in SPR sensors to detect molecular binding events, making it valuable in fields like biology, chemistry, and medicine.
6. Real-Time Monitoring:
– Changes in the refractive index, caused by molecular interactions, lead to shifts in the SPR angle, allowing real-time monitoring of binding events.
7. Label-Free Detection:
– One of SPR’s significant advantages is its label-free nature, eliminating the need for fluorescent or radioactive tags in biological sensing.
8. Biomedical and Environmental Applications:
– Widely applied in studying biomolecular interactions, drug discovery, and environmental monitoring due to its high sensitivity and specificity.
In summary, SPR is a powerful technique that leverages the interaction between light and surface plasmons to enable precise and real-time detection of molecular events, making it a cornerstone in various scientific and medical research applications.
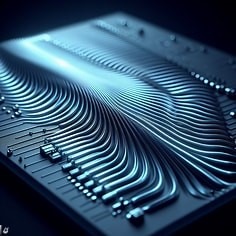
Graphene Plasmonics
Graphene plasmonics is an exciting and rapidly evolving field that explores the unique plasmonic properties of graphene, a single layer of carbon atoms arranged in a hexagonal lattice. Here’s an overview:
1. Graphene Basics:
– Graphene is an excellent conductor of electricity, and its single-atom thickness makes it an ideal material for plasmonic applications.
2. Plasmonic Behavior:
– When light interacts with graphene, it can induce collective oscillations of electrons, known as graphene plasmons.
3. Tunable Plasmons:
– The plasmonic properties of graphene can be dynamically tuned by adjusting factors like the carrier concentration, allowing for versatile control of light-matter interactions.
4. Hyperbolic Dispersion:
– Graphene exhibits hyperbolic dispersion in the terahertz and mid-infrared frequencies, enabling unique plasmonic behavior not found in traditional materials.
5. Enhanced Light-Matter Interaction:
– Graphene plasmons can concentrate electromagnetic fields on the nanoscale, leading to enhanced light-matter interactions. This is valuable for applications in sensing and imaging.
6. Integration with Other Materials:
– Graphene can be combined with other plasmonic materials or structures to create hybrid systems with enhanced and tunable plasmonic responses.
7. Applications:
– Sensing: Graphene plasmonics is employed in ultrasensitive sensors, detecting small changes in the environment, including gas molecules or biomolecules.
– Imaging: It contributes to the development of high-resolution imaging devices, especially in the terahertz and infrared spectral ranges.
– Optoelectronics: Graphene plasmonics holds promise for advanced optoelectronic devices, such as modulators and photodetectors.
8. Challenges and Future Directions:
– Challenges include minimizing losses and developing scalable fabrication methods. Future directions involve exploring quantum aspects of graphene plasmons and further integration into practical devices.
In essence, graphene plasmonics combines the unique properties of graphene with plasmonic principles, opening up new possibilities for the development of advanced technologies with applications ranging from sensing to optoelectronics.
How Surface Plasmon Resonance (SPR) Works
Surface Plasmon Resonance is a phenomenon that occurs at the interface of a thin metal film, commonly gold or silver, and a surrounding medium (usually a dielectric). It is widely used in sensing applications, and here’s how it works:
1. Metal-Dielectric Interface:
– SPR takes place at the surface of a metal film, where there is an interaction between the metal and the adjacent dielectric material.
2. Incident Light Angle:
– A beam of light is directed onto the metal surface at a specific angle known as the incident angle.
3. Electron Excitation:
– At the incident angle, the energy from the light matches the energy required to excite surface plasmons – collective oscillations of free electrons at the metal surface.
4. Resonance Condition:
– When the resonance condition is met, the energy from the incoming light is transferred to the surface plasmons, leading to their excitation.
5. Refractive Index Sensitivity:
– The resonance condition is highly sensitive to changes in the refractive index of the medium adjacent to the metal surface.
6. Binding Events Detection:
– When molecules bind to the metal surface or the dielectric medium undergoes changes (due to, for example, biomolecular interactions), the refractive index at the metal-dielectric interface changes.
7. Shift in Resonance Angle:
– This change in refractive index causes a shift in the angle at which resonance occurs, known as the SPR angle shift.
8. Real-Time Monitoring:
– By monitoring this shift in real-time, scientists can detect and quantify binding events without the need for labels or markers, making SPR a powerful and label-free sensing technique.
9. Applications:
– SPR is widely used in various fields, including biology, chemistry, and medicine, for studying molecular interactions, detecting biomolecules, and monitoring binding events.
In summary, SPR relies on the precise interaction between light and surface plasmons at a metal-dielectric interface, providing a sensitive and real-time method for detecting changes in the surrounding medium, making it a key technology in biosensing and other analytical applications.
Surface Plasmon Resonance Instruments
Surface Plasmon Resonance (SPR) instruments are sophisticated tools designed to exploit the SPR phenomenon for various applications, particularly in biosensing and material science. Here’s an overview of key components and features commonly found in SPR instruments:
1. Light Source:
– SPR instruments typically use a monochromatic light source, often a laser, to generate a beam of light for interaction with the metal-dielectric interface.
2. Prism or Sensor Chip:
– The metal-dielectric interface is usually created on a prism or a sensor chip, commonly made of materials like glass coated with a thin layer of gold or silver.
3. Flow Cell or Sample Chamber:
– A flow cell or sample chamber allows the controlled flow of the analyte or sample over the metal-dielectric interface, facilitating interaction with the surface and enabling real-time monitoring.
4. Detector:
– Detectors capture the reflected light from the metal-dielectric interface. Changes in the intensity of the reflected light are indicative of shifts in the SPR angle, reflecting changes in the sample.
5. Microfluidic System:
– Many modern SPR instruments incorporate microfluidic systems for precise and automated sample delivery. This ensures accurate and controlled interactions between the sample and the metal surface.
6. Temperature Control:
– Temperature control is crucial for maintaining stability in the experiment. Many SPR instruments have built-in systems to regulate temperature during measurements.
7. Software for Data Analysis:
– Specialized software processes the data obtained during SPR experiments. This includes analyzing the SPR angle shifts and providing output graphs and results.
8. Automation and High-Throughput Systems:
– Advanced SPR instruments often include automation features and high-throughput capabilities, allowing for the analysis of multiple samples in parallel.
9. Multi-Channel Systems:
– Some instruments have multiple channels, enabling the simultaneous study of different interactions or the screening of multiple samples.
10. Integration with Other Techniques:
– SPR instruments may be integrated with complementary techniques such as mass spectrometry or fluorescence for comprehensive analysis.
11. Portable SPR Devices:
– There is a trend towards developing portable SPR devices, suitable for on-site or point-of-care applications, expanding the accessibility of SPR technology.
Plasmonic Materials
Plasmonic materials are substances that exhibit unique optical properties related to the excitation of surface plasmons – collective oscillations of free electrons – when interacting with light. These materials play a crucial role in the field of plasmonics, enabling the manipulation and control of light at the nanoscale. Some key plasmonic materials include:
1. Noble Metals:
– Gold and Silver: Among the most widely used plasmonic materials due to their excellent ability to support surface plasmons in the visible and near-infrared spectrum.
2. Aluminum:
– An alternative to gold and silver, especially in the ultraviolet range. Aluminum supports plasmonic resonances and is more cost-effective.
3. Copper:
– Exhibits plasmonic behavior in the visible and near-infrared regions. However, its tendency to oxidize can be a challenge.
4. Graphene:
– A single layer of hexagonally latticed carbon atoms. Graphene plasmons offer tunable and highly sensitive responses, particularly in the terahertz and mid-infrared regions.
5. Semiconductor Materials:
– Indium Tin Oxide (ITO): Used in the infrared range for its plasmonic characteristics.
– Gallium Arsenide (GaAs): Exhibits plasmonic behavior in the near-infrared.
6. Dielectric Materials:
– Silicon and Silicon Nitride: Although traditionally considered insulators, under certain conditions, they can support plasmonic resonances.
– Titanium Dioxide (TiO2): Exhibits plasmonic properties in the ultraviolet range.
7. Hybrid Materials:
– Combinations of metals and dielectrics to create structures with tailored plasmonic responses. These hybrids allow for enhanced control and manipulation of plasmons.
8. Two-Dimensional Materials:
– Graphene: Apart from its plasmonic properties, graphene also serves as a building block for other two-dimensional plasmonic materials.
9. Metamaterials:
– Engineered materials with unique properties not found in nature. Metamaterials enable precise control over plasmonic responses, leading to applications like superlensing and cloaking.
10. Organic Materials:
– Some organic molecules and polymers exhibit plasmonic behavior, offering opportunities for plasmonics in bio-sensing and optoelectronics.
Understanding and manipulating the plasmonic properties of these materials allow scientists and engineers to design advanced devices for applications such as sensors, imaging, and energy harvesting at the nanoscale.
Plasmonic Nanoparticles
Plasmonic nanoparticles are tiny particles, typically on the nanometer scale, made from materials that exhibit plasmonic behavior. These nanoparticles interact strongly with light, thanks to the excitation of surface plasmons, and find diverse applications in various fields. Here’s an overview:
1. Materials:
– Plasmonic nanoparticles are often made from noble metals such as gold and silver due to their excellent plasmonic properties. Other materials, including aluminum and copper, are also explored for specific applications.
2. Size and Shape:
– The size and shape of plasmonic nanoparticles strongly influence their optical properties. Shapes like spheres, rods, and triangles exhibit different plasmonic resonances, allowing for tailored applications.
3. Plasmon Resonance:
– Plasmonic nanoparticles exhibit a phenomenon known as localized surface plasmon resonance (LSPR). This resonance occurs when the frequency of incident light matches the natural frequency of the nanoparticle’s surface plasmons.
4. Enhanced Light-Matter Interaction:
– Due to LSPR, plasmonic nanoparticles can concentrate and enhance local electromagnetic fields. This property is harnessed for applications in sensing, imaging, and light manipulation.
5. Applications:
– Sensing: Plasmonic nanoparticles are used as sensing probes in various applications, detecting changes in the surrounding environment based on shifts in their plasmonic resonance.
– Imaging: In biomedical imaging, plasmonic nanoparticles enhance contrast and enable high-resolution imaging, contributing to diagnostics and research.
– Catalysis: Plasmonic nanoparticles can act as catalysts in chemical reactions, utilizing their unique optical and electronic properties for enhanced catalytic performance.
– Therapeutics: In photothermal therapy, plasmonic nanoparticles convert absorbed light into heat, selectively destroying targeted cells for therapeutic purposes.
– Energy Harvesting: Plasmonic nanoparticles are employed in solar cells to enhance light absorption and improve energy conversion efficiency.
6. Surface Functionalization:
– Plasmonic nanoparticles can be functionalized with various molecules, such as antibodies or ligands, for specific interactions with biological targets. This is particularly useful in biosensing and targeted drug delivery.
7. Colloidal Stability:
– Colloidal stability is crucial for the practical use of plasmonic nanoparticles. Surface modifications and coatings are often applied to prevent aggregation and ensure stability in different environments.
8. Quantum Plasmonics:
– Quantum aspects of plasmonic nanoparticles are explored for quantum information processing and quantum-enhanced sensing applications. In summary, plasmonic nanoparticles offer a versatile platform for manipulating light at the nanoscale, leading to a wide range of applications in fields such as medicine, electronics, energy, and environmental monitoring.
Plasmonics Applications
Plasmonics, with its ability to manipulate light at the nanoscale, has found diverse applications across various scientific and technological fields. Here are some notable applications:
1. Sensing and Detection:
– Biosensors: Plasmonic sensors can detect biomolecular interactions in real-time, enabling applications in medical diagnostics, environmental monitoring, and food safety.
– Chemical Sensing: Plasmonic devices are used to identify and quantify chemical substances, offering high sensitivity and specificity.
2. Imaging and Microscopy:
– Super-Resolution Microscopy: Plasmonics contributes to achieving resolutions beyond the diffraction limit in microscopy, allowing for detailed imaging of biological structures at the nanoscale.
– In Vivo Imaging: Plasmonic nanoparticles enhance contrast and resolution in biomedical imaging, aiding in the visualization of tissues and cellular structures.
3. Optoelectronics:
– Plasmonic Circuits: Integration of plasmonic components in electronic circuits for ultra-compact and high-speed information processing.
– Photodetectors: Plasmonic materials enhance light absorption and detection capabilities in photodetectors, improving efficiency.
4. Energy Harvesting:
– Solar Cells: Plasmonic nanoparticles enhance light absorption in solar cells, leading to increased energy conversion efficiency.
– Thermoplasmonics: Plasmonic materials can convert absorbed light into heat, utilized for localized heating and energy harvesting applications.
5. Communication Technologies:
– Plasmonic Waveguides: Enable the transmission of information at the nanoscale, contributing to the development of high-speed and compact communication devices.
– Plasmonic Antennas: Enhance signal strength and directionality in wireless communication systems.
6. Therapeutics:
– Photothermal Therapy: Plasmonic nanoparticles can absorb light and convert it into heat, enabling targeted destruction of cancer cells in photothermal therapy.
– Drug Delivery: Plasmonic materials are employed in controlled drug release systems for targeted delivery to specific tissues.
7. Metamaterials and Cloaking:
– Invisibility Cloaks: Metamaterials with plasmonic properties are explored for creating cloaking devices that can manipulate the path of light around objects.
– Negative Refraction: Plasmonic metamaterials enable negative refraction, influencing the behavior of light in unique ways.
8. Quantum Plasmonics:
– Quantum Information Processing: Exploring the quantum aspects of plasmons for the development of quantum-enhanced information processing devices.
9. Light Emission:
– Plasmon-Enhanced Light Emission: Plasmonic structures can enhance light emission in devices such as light-emitting diodes (LEDs) and lasers.
10. Environmental Monitoring:
– Gas and Chemical Sensing: Plasmonic sensors are used for detecting and monitoring pollutants, contributing to environmental monitoring and safety. These applications highlight the versatility of plasmonics in advancing technologies across different disciplines, promising innovations with far-reaching implications.
Challenges
1. Losses and Heating:
– Plasmonic materials often suffer from energy losses, leading to heating effects. Overcoming these losses is crucial for applications such as photothermal therapy and energy harvesting.
2. Fabrication Techniques:
– Precise fabrication of plasmonic nanostructures poses challenges. Developing scalable and cost-effective techniques for creating well-defined structures is essential for widespread use.
3. Integration with Other Technologies:
– Integrating plasmonic devices with existing technologies can be challenging. Compatibility and efficient coupling with electronic and optoelectronic systems need further exploration.
4. Quantum Plasmonics:
– Understanding and controlling the quantum aspects of plasmons, known as quantum plasmonics, pose challenges. This includes maintaining coherence and addressing quantum decoherence effects.
5. Environmental Impact:
– Some plasmonic materials, particularly noble metals, can have environmental impacts. Finding environmentally friendly alternatives or mitigating these effects is a consideration.
6. Biocompatibility:
– In biomedical applications, ensuring the biocompatibility of plasmonic materials is crucial. Addressing potential toxicity concerns is essential for safe and effective use in medical settings.
7. Loss of Localized Surface Plasmon Resonance (LSPR) Control:
– Maintaining control over LSPR in complex environments, such as biological systems, can be challenging due to variations in refractive index and other factors.
Future Prospects
1. Advanced Nanofabrication Techniques:
– Progress in nanofabrication methods, including techniques like electron-beam lithography and nanoimprint lithography, will enhance the precision and scalability of plasmonic device manufacturing.
2. Quantum Plasmonics Advancements:
– Exploring and harnessing the quantum aspects of plasmons could lead to the development of quantum-enhanced technologies, including quantum information processing and quantum sensors.
3. Hybrid Plasmonic Systems:
– Integrating plasmonic materials with other materials, such as 2D materials and semiconductors, can result in hybrid systems with enhanced and tunable plasmonic responses.
4. Active Plasmonics:
– Developing materials with dynamically tunable plasmonic properties, known as active plasmonics, offers opportunities for real-time control and modulation of plasmonic effects.
5. Sustainable Plasmonics:
– Research into sustainable plasmonic materials and methods, such as exploring earth-abundant materials, can address environmental concerns associated with current plasmonic technologies.
6. Biomedical Breakthroughs:
– Advancements in plasmonic technologies for medical applications, including targeted drug delivery, imaging, and diagnostics, hold great promise for improving healthcare outcomes.
7. Ultrafast Plasmonics:
– Progress in ultrafast plasmonics, enabling the manipulation of plasmons on extremely short timescales, opens avenues for high-speed communication and signal processing.
8. Innovative Energy Harvesting:
– Further exploration of plasmonic materials for energy harvesting applications, such as plasmon-enhanced photovoltaics and thermoelectric devices, could contribute to sustainable energy solutions.
Conclusion
In conclusion, plasmonics is a captivating field that unlocks the extraordinary potential of manipulating light on a tiny scale. By harnessing the collective dance of electrons, known as surface plasmons, researchers have paved the way for groundbreaking applications. Plasmonic materials, like gold and silver nanoparticles, act as tiny superheroes, enabling advancements in sensing, imaging, energy, and beyond. Challenges, such as losses and environmental considerations, remain, but ongoing research promises exciting solutions. As we navigate the frontiers of nanotechnology, the future of plasmonics holds the key to innovations that could revolutionize fields from medicine to communication, making the invisible dance of electrons a powerful force in shaping our technological landscape.
Frequently Asked Questions:
Q: What is Surface Plasmon Resonance?
A: Surface Plasmon Resonance (SPR) is a phenomenon occurring at the interface of a thin metal film and a dielectric material, typically used in biosensors. When light hits this interface at a specific angle, it excites collective oscillations of free electrons, known as surface plasmons. The resonance condition is highly sensitive to changes in the refractive index of the adjacent medium. By monitoring shifts in the resonance angle in real-time, SPR enables label-free detection of molecular binding events, making it a powerful tool in fields such as biotechnology and medical diagnostics.